Lasers have revolutionized numerous fields of science and society, serving as versatile light sources that have transformed scientific investigations and powered trillion-dollar industries. In manufacturing, programmable robotic lasers have surpassed traditional mechanical tools, offering capabilities like cutting, drilling, measuring, and even welding and peening—all from a single laser station. In the military, lasers play crucial roles in communications, remote sensing, directed energy, and materials production and diagnosis in extreme environments.
In the realm of ultrafast science, attosecond lasers have emerged as a groundbreaking technology, enabling researchers to explore the quantum world with unprecedented precision. With pulse durations on the scale of attoseconds (one quintillionth of a second), these lasers open new frontiers in understanding and manipulating matter at the most fundamental levels. This article delves into the technology behind attosecond lasers, their current applications, and future prospects in various scientific and technological domains.
The Evolution of Ultrafast Lasers
Among the various types of lasers, mode-locked lasers are notable for emitting ultrashort pulses, typically in the femtosecond (one quadrillionth of a second) to picosecond (one trillionth of a second) range. These ultrafast lasers utilize processes that enable rapid changes in optical power, with pulse repetition rates often around 100 MHz, though they can range from a few MHz to several GHz. However, the quest for even shorter pulses has led scientists to develop attosecond lasers, pushing the boundaries of time measurement and control.
Attosecond Pulses: The Frontier of Time Measurement
Physicists and engineers aim to understand the movements of electrons and subatomic particles, requiring precision in the attosecond (one quintillionth of a second) and zeptosecond (one sextillionth of a second) range. Achieving such precision involves producing pulses with higher-wavelength sources and broader frequency ranges. Attosecond lasers are the epitome of ultrafast laser technology. Producing pulses that last only a few attoseconds, they allow scientists to capture and study electron dynamics in real time.
The shortest pulse measured to date is 80 attoseconds, a milestone achieved through various methods, including large-scale electron accelerators like the Stanford Linear Accelerator. Attosecond science began with the nonlinear compression of intense laser pulses to durations below two optical cycles, and creating such short pulses still necessitates state-of-the-art few-cycle laser amplifiers.
The creation of such short pulses involves several sophisticated techniques:
High-Harmonic Generation (HHG): A Breakthrough in Ultrafast Optics
Attosecond pulses are typically generated through a process called high-harmonic generation. In HHG, a high-intensity femtosecond laser pulse is focused into a gas, causing the gas atoms to emit light at multiples (harmonics) of the original laser frequency. By carefully isolating the appropriate harmonics, researchers can produce attosecond pulses.
High-harmonic generation (HHG) is a critical process in creating attosecond pulses. A further conceptual breakthrough in ultrafast optics was achieved with the discovery of high-harmonic generation (HHG) from noble gas atoms illuminated by ultrashort light pulses with a peak intensity of the order of 1013-1015 W/cm2 . The process of HHG can be intuitively understood in terms of the so-called “three-step model”
- Ionization: An intense laser field ionizes an electron from an atom, commonly a noble gas, and accelerates it away.
- Acceleration: The oscillating electric field changes direction, causing the electron to accelerate back towards the parent ion, gaining kinetic energy.
- Recombination: The electron recombines with the parent ion, releasing excess kinetic energy as a high-energy photon, often in the VUV to soft X-ray region.
This cycle repeats every half-cycle of the driving laser, generating an attosecond pulse train. To isolate a single attosecond pulse, various optical gating techniques modify the ionization, acceleration, or recombination steps.
Phase Matching:
- Achieving efficient HHG requires phase matching, where the phase velocity of the generated harmonics matches that of the driving laser pulse. This ensures coherent addition of the emitted harmonics, leading to the generation of high-intensity attosecond pulses.
Pulse Compression:
- Techniques like chirped pulse amplification and pulse compression are used to shorten the laser pulses to the attosecond regime. These methods involve stretching the laser pulse in time, amplifying it, and then compressing it back to a shorter duration.
Generating Isolated Attosecond Pulses
For many applications, it is beneficial to generate isolated attosecond pulses rather than a train of pulses. This can be achieved through various optical gating techniques that modify the steps in the three-step model:
- Ionization Gating: A single field maximum of the driving laser pulse ionizes a significant portion of the atoms, leading to electron acceleration and recombination that generates an attosecond pulse. Subsequent cycles either lack enough electrons for ionization or have unsuitable phase matching conditions due to the plasma created, resulting in a single attosecond pulse.
- Amplitude Gating: The strongest field maximum accelerates the photoionized electrons most effectively, generating the highest-energy photons. Filtering out the spectral components below the cutoff generated by weaker maxima ensures that the remaining spectrum originates from the same attosecond burst, producing a single pulse.
- Polarization Gating: The laser field’s ellipticity varies with time, creating a single linearly-polarized field maximum surrounded by elliptically-polarized fields. Electrons accelerated by the elliptically-polarized fields do not recombine with the parent ion, resulting in only the electrons from the linearly-polarized maximum producing high-energy photons, thereby generating a single attosecond pulse.
Combining Gating Methods
Combining different gating methods can enhance the generation of isolated attosecond pulses. For instance, double optical gating combines polarization gating and two-color gating (an ionization-type gating) to relax the constraints on the driving laser. Other methods include wavefront-based gating techniques such as the attosecond lighthouse and noncollinear optical gating, which produce an attosecond pulse train with angularly-resolvable pulses.
Each gating technique has its advantages and disadvantages, including requirements for pulse duration, carrier-envelope phase stability, achievable spectrum, and the need for appropriate filters. Understanding these methods provides a foundational introduction to the field of attosecond science.
Applications of Attosecond Lasers
Since the first generation of attosecond pulses in 2001, these ultrafast pulses have revolutionized time-resolved spectroscopy, enabling direct observation and control of electronic dynamics in atoms, molecules, nanostructures, and solids.
Since their first experimental demonstration in 2001, attosecond pulses have revolutionized time-resolved spectroscopy, offering unparalleled temporal resolution. The primary goal of attosecond science is to gain direct access to electronic dynamics in atoms, molecules, nanostructures, and solids, enabling control over these ultrafast processes. Over the past two decades, attosecond pulses have been applied to a wide range of fields, from atomic physics to solid-state physics, yielding numerous groundbreaking discoveries.
Atomic Physics
In atomic physics, attosecond techniques have been crucial for measuring the delay in photoemission and analyzing tunnel ionization processes. These measurements provide deeper insights into the interactions and behaviors of electrons under extreme conditions.
Molecular Physics
The use of attosecond pulses in molecular physics began in 2010, with pioneering investigations into charge localization processes in hydrogen molecules. More recently, attosecond pulses have been employed to study charge migration in amino acids. Charge migration refers to the ultrafast movement of charge within a molecule, driven by electron correlations, which can occur on timescales ranging from a few femtoseconds to tens of attoseconds. For example, the first experimental measurement of charge migration in the α-amino acid phenylalanine (C9H11NO2) following attosecond excitation was reported in 2014.
Phenylalanine, an aromatic amino acid, plays a crucial role in the biosynthesis of other amino acids and is essential for the structure and function of many proteins and enzymes. Understanding electron dynamics in such biologically relevant molecules can open new research frontiers, revealing how sub-femtosecond electron processes determine the fate of biomolecules.
Solid-State Physics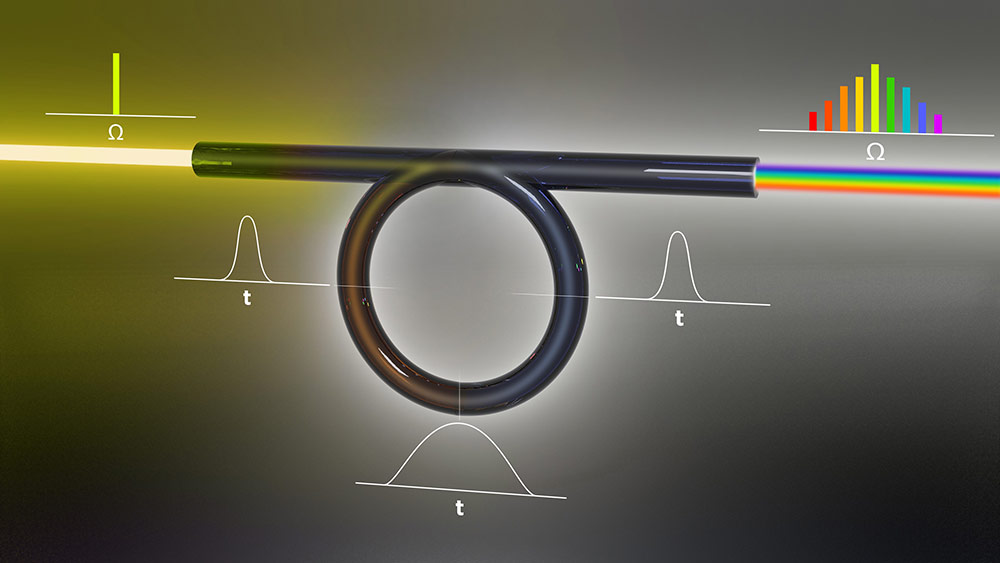
Though still in its early stages, attosecond science has already yielded significant results in solid-state physics. Attosecond techniques allow researchers to investigate physical processes in solids that are inaccessible with longer temporal resolutions. Key findings include:
- Photoemission Delays: Measurements of photoemission delays in various solids, which are on the order of tens of attoseconds.
- Intra-Band Charge Motion: Observation of the intra-band charge motion leading to the Franz-Keldysh effect in dielectrics, evolving on attosecond timescales.
- Carrier-Induced Band-Gap Reduction: Determination of the upper limit for carrier-induced band-gap reduction and electron-electron scattering time in the conduction band of silicon, both on the order of a few hundred attoseconds.
These results highlight the significant advantages of applying attosecond techniques to solid-state physics, enabling the exploration of ultrafast electronic processes in materials.
Advanced Methods and Accessibility
Recent advancements in generating intense ultrashort pulses in the VUV/X-ray energy region, such as X-ray free-electron lasers (FELs), have further expanded the capabilities of attosecond science. Techniques developed over the past years aim to generate high-energy attosecond pulses, enhancing the potential for new discoveries.
Researchers at the University of Central Florida (UCF) have made significant strides in making attosecond science more accessible. Their innovative methods, detailed in a study published in Science Advances in 2020, involve using industrial-grade lasers to generate attosecond pulses. This approach simplifies the generation of ultrashort pulses and reduces the complexity and cost associated with traditional setups.
By compressing longer laser pulses using molecular gases like nitrous oxide, UCF researchers have demonstrated pulse compression to just 1.6 cycles, with single-cycle pulses within reach. This development reduces the reliance on expensive, complex laser systems and makes attosecond science more approachable for researchers across various scientific disciplines.
The unique capabilities of attosecond lasers have opened up a plethora of applications across various scientific fields:
1. Observing Electron Dynamics:
- Attosecond lasers enable the direct observation of electron movements within atoms and molecules. This capability is crucial for understanding fundamental processes such as chemical reactions, ionization, and charge transfer.
2. Ultrafast Spectroscopy:
- In ultrafast spectroscopy, attosecond pulses are used to study the behavior of electrons and atoms on their natural timescales. This technique provides insights into the electronic structure and dynamics of complex materials, contributing to advancements in fields like photovoltaics and semiconductor technology.
3. Attosecond Metrology:
- Attosecond pulses serve as precise probes for time-resolved measurements, allowing scientists to measure time intervals with attosecond accuracy. This is vital for developing highly accurate atomic clocks and enhancing our understanding of fundamental physical constants.
4. Quantum Control:
- Attosecond lasers facilitate the manipulation of quantum states, enabling control over electron wave packets. This has potential applications in quantum computing and information processing, where precise control over quantum bits (qubits) is essential.
5. Medical Imaging and Therapy:
- The high precision and energy of attosecond pulses could revolutionize medical imaging techniques, offering non-invasive methods to probe biological tissues at the cellular and molecular levels. Additionally, attosecond lasers hold promise for advanced therapeutic applications, such as targeted cancer treatments.
Future Prospects
The future of attosecond technology is bright, with ongoing research aimed at enhancing pulse energy, integrating with other advanced technologies, and making attosecond science more accessible. Researchers at the University of Central Florida (UCF) have developed methods to generate attosecond pulses using industrial-grade lasers, making this cutting-edge field more accessible to researchers across various disciplines. By compressing longer laser pulses with molecular gases, such as nitrous oxide, they have simplified the generation of ultrashort pulses, reducing complexity and cost.
1. Higher Energy Pulses:
- Efforts are underway to increase the energy of attosecond pulses, enabling deeper and more detailed investigations of matter. Higher energy pulses will open new avenues in materials science and high-energy physics.
2. Integration with Other Technologies:
- Combining attosecond lasers with other cutting-edge technologies, such as X-ray free-electron lasers (XFELs) and synchrotrons, will provide synergistic benefits. This integration will enhance the resolution and scope of ultrafast investigations.
3. Portable Attosecond Sources:
- Developing compact and portable attosecond laser systems will make this technology more accessible to a broader range of laboratories and industries. This democratization of attosecond technology could spur innovation across multiple fields.
4. Industrial Applications:
- Attosecond lasers have the potential to revolutionize industrial processes, such as precision manufacturing and material processing. Their ability to deliver ultra-short, high-intensity pulses can lead to the development of new techniques for cutting, drilling, and surface modification with unprecedented precision.
The Future of Attosecond Technology
The future of attosecond technology is promising, with ongoing research aimed at enhancing pulse energy, integrating with other advanced technologies, and making the technology more accessible:
- Higher Energy Pulses: Increasing pulse energy will enable deeper and more detailed investigations in various scientific fields.
- Integration with Other Technologies: Combining attosecond lasers with X-ray free-electron lasers (XFELs) and synchrotrons will enhance ultrafast investigations.
- Portable Attosecond Sources: Developing compact, portable attosecond laser systems will democratize access to this technology, spurring innovation across multiple disciplines.
- Industrial Applications: Attosecond lasers could revolutionize precision manufacturing, offering new techniques for cutting, drilling, and surface modification.
Promising Research Directions
Looking ahead, several exciting areas of research hold immense potential for attosecond lasers:
- Attosecond Coherent Light Sources: Developing stable and coherent attosecond light sources would enable new avenues for studying complex light-matter interactions.
- Attosecond Metrology: Attosecond lasers could lead to the development of ultra-precise attosecond clocks and measurement tools, pushing the boundaries of timekeeping and synchronization.
- Attosecond Manipulation of Materials: The ability to control electron dynamics on the attosecond timescale could open doors for manipulating material properties at will, with implications for electronics, photonics, and other fields.
Latest Breakthroughs in Attosecond Lasers
The field of attosecond science is experiencing a period of rapid advancement. Here’s a breakdown of the latest breakthroughs pushing the boundaries of attosecond laser technology and its applications:
Record-Breaking Pulse Amplification:
- Higher Peak Power: Researchers at RIKEN, Japan, recently achieved a breakthrough by amplifying single-cycle attosecond pulses to over 50 millijoules, exceeding previous records by a factor of 50. This immense energy translates to peak powers in the terawatt (trillion watts) range, enabling even deeper exploration of electron dynamics.
Enhanced Repetition Rates:
- Faster Sampling: Traditionally, attosecond lasers operated at low repetition rates, limiting the amount of data collected. A recent development by an international team achieved a repetition rate of 1 kilohertz (1,000 pulses per second) for attosecond pulses. This significant improvement allows for faster and more comprehensive studies of ultrafast phenomena.
New Approaches to Pulse Generation:
- Beyond Traditional Techniques: New methods for generating attosecond pulses are emerging, offering potential advantages. Techniques like laser-driven wakefield acceleration and high-harmonic generation using hollow-core fibers are being explored for their efficiency and potential for scalability.
Improved Synchronization Techniques:
- Precise Timing is Key: Studying events on the attosecond timescale requires precise synchronization between the attosecond laser pulse and other tools used in the experiment. Recent advancements in synchronization techniques are improving the accuracy and reliability of attosecond measurements.
Integration with Artificial Intelligence (AI):
- AI-powered Optimization: AI algorithms are being explored to analyze and optimize attosecond laser pulse generation and characterization. This collaboration between human expertise and machine learning has the potential to accelerate discoveries in attosecond science.
Making Attosecond Science Accessible
Researchers at the University of Central Florida (UCF) have made significant strides in making attosecond science more accessible. By using industrial-grade lasers and innovative techniques, they have simplified the generation of attosecond pulses, enabling interdisciplinary applications.
Their approach involves compressing longer laser pulses using molecular gases, like nitrous oxide, in place of noble gases. This method allows the production of ultrashort pulses from commercially available lasers, reducing complexity and cost.
Conclusion
Attosecond lasers represent a monumental leap in our ability to observe and control the quantum world. Their ability to generate the shortest pulses of light ever created has profound implications for science and technology. Their unparalleled precision opens new frontiers in science and technology, promising advancements in fields ranging from fundamental physics to industrial manufacturing.
As research continues to advance this field, the applications and impact of attosecond lasers will undoubtedly expand, driving innovation and discovery across a multitude of disciplines. The future of attosecond laser technology is not just about seeing the world in a new light, but about shaping it in ways we are only beginning to imagine.